Wavefront sensing and control are essential for high-contrast imaging systems. Aberrations [kun je dit specificeren? ‘light’ aberrations bijvoorbeeld?] created by the Earth’s atmosphere, the telescope itself, or optics in the instrument reduce the performance and must be corrected. A wavefront sensor measures the distortion in the wavefront.
The LEOPARD group puts continuous effort into developing improved wavefront sensors, control algorithms, and wavefront sensing algorithms that use the science focal-plane. [graag iets verhelderen wat de science focal-plane? dat is het primaire beeld van de telescoop? ‘focal plane’ komt veel voor in deze tekst, dus wel belangrijk dat dit helder is voor de lezer. misschien kunnen we het aangeven in het figuur?]
A distorted wavefront (top) is corrected via a wavefront sensor (bottom) that controls an adaptive mirror. [dit figuur lijkt mij verhelderend, het stond bij de media in het CMS. ken jij toevallig de credits voor dit figuur?]
Wavefront sensors
Thinking of creative ways to make use of liquid crystal technologies (LC), the LEOPARD group has developed several wavefront sensors including the vector Zernike wavefront sensor and the generalized optical differential wavefront sensor. We can also combine a coronagraph with wavefront sensing resulting in the coronagraphic Modal WFS. Here we will take a closer look at these three technologies.
The Zernike wavefront sensor (vZWFS) is a natural progression from the Zernike WFS [link naar meer informatie? of een korte uitleg? als lezer weet ik niet wat dit inhoud] as a result of liquid crystal technology. Due to the induced polarization by LC, we are able to split the light into two polarized states [verticaal versus horizontaal, links- rechtsdraaiend?] resulting in two pupil images directly encoding the electric field of the incoming aberrated wavefront. [waarom is het belangrijk om dat te weten?] The two pupils [even uitleggen wat een ‘pupil’ is, een beeld?] allow the vZWFS to estimate not only the phase but also the amplitudes resulting in knowing the complete electric field. [en dus hoe het wavefront verstoord is?] The vZWFS is currently being tested [misschien dat stukje over testen schrappen? dat veroudert wellicht snel] in collaboration with the Jet Propulsion Laboratory for use on future space telescopes like HabEx and LUVIOR.
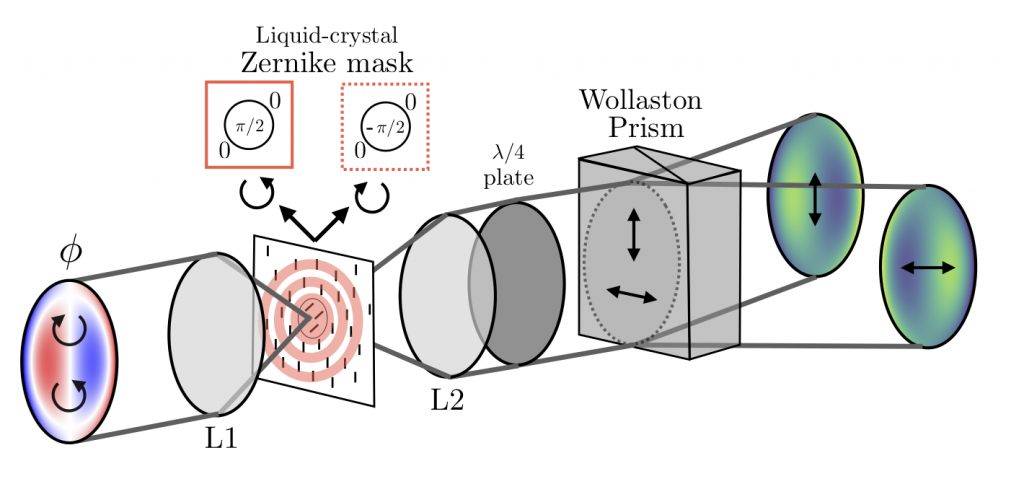
The generalized optical differential wavefront sensor (g-ODWFS) allows for a variety of different wavefront sensor types (from the pyramid wavefront sensor to the optical differential WFS) to be created using a amplitude mask in which the gradient of the mask changes the dynamic range and sensitivity of the WFS. [voor mij gaat dit te snel. wat doet het amplitude masker? licht volledig blokkeren? en waarom is dat gradiënt van belang?] Currently, the LEXI instrument makes use the g-ODWFS.
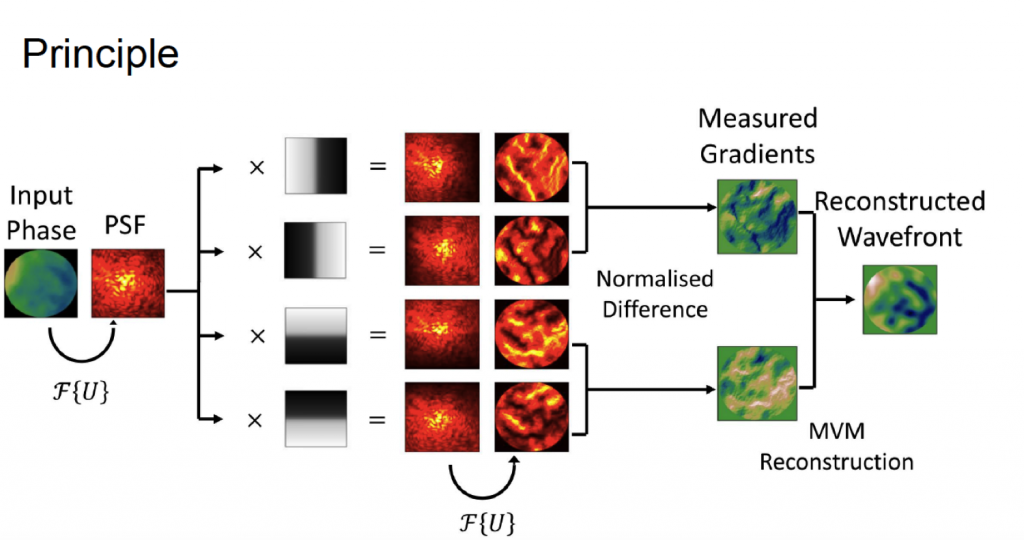
The Modal WFS (cMWS) is made by incorporate holographic spots [ik weet wel wat die holografische spots zijn, maar de gemiddeld lezer niet… is daar een synoniem voor of kun je het beschrijven?] on the same liquid crystal phase plate as our coronagraphs. The holographic spots are ‘copies’ of the the light source within the image [ik probeer het even uit te leggen… zoiets OK? en anders moet je PSF weer uitleggen…] that are artificially biased with a set of aberration modes (for example Zernike modes [wat is een Zernike mode? anders een link toevoegen?]). We can then choose an arbitrary number of modes to fill the focal plane. This naturally leads to another implementation of phase diversity where we have one set holographic spots. [de zin hiervoor snap ik niet… wat is die andere implementatie dan?] This WFS enables wavefront sensing in the focal plane [betekent dat vóór de spiegel?] and is suitable for real-time non-common-path-error correction. [is dit dan per definitie moeilijk of onmogelijk met andere WFSs?]
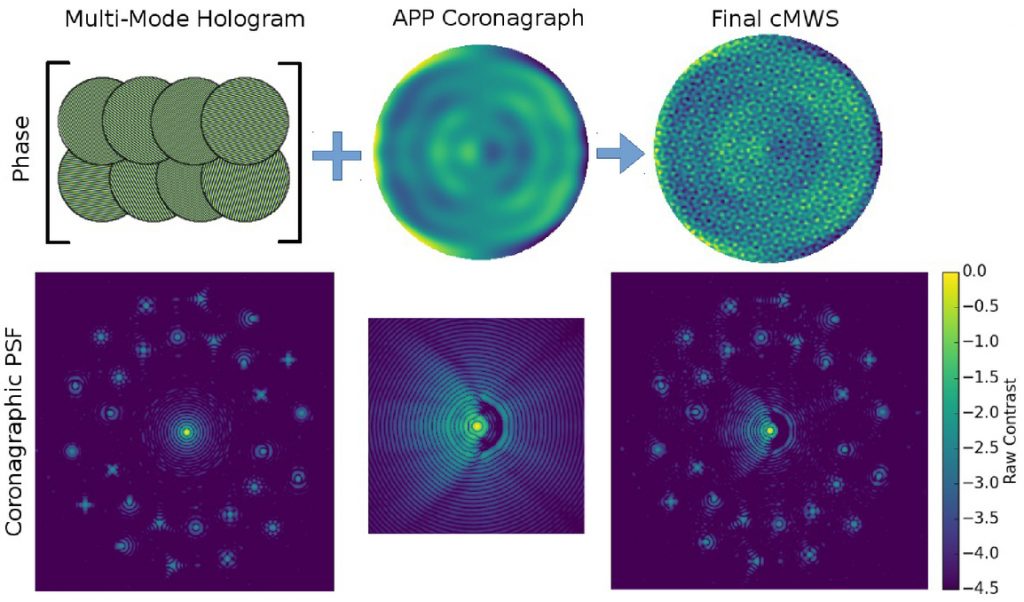
Wavefront control
We have many different on-going research happening in wavefront control related to improve the contrast for direct imaging. [ik zou hier liever kort lezen wat het wavefront control ís, kort door de bocht een computerprogramma dat de adaptieve optiek aanstuurt op basis van info van de WFS?]
LEOPARD scientists work on simulations for linear prediction to reduce the servo-lag error [graag kort uitleggen]. The research also spans modelling of non-stationary behaviour of atmospheric wavefront fluctuations. Simple Prediction schemes such a linear minimum mean square error prediction as well as adaptive implementations have shown to be very sensitive to wind speed fluctuations in the atmosphere. Further work into reducing the sensitivity and tracking these changes is being done.
Another area of control research being explored is machine learning for high contrast imaging. More specifically, using the raw contrast [bedoel je hier het contrast van het telescoopbeeld?] as a ‘reward function’ to drive the learning algorithms. In this work we combine not only wavefront sensing data but also focal plane information to help improve the contrast.
Focal-plane wavefront sensing
One of the current limitations in high-contrast imaging are non-common path aberrations (NCPA) [kun je benoemen wat er dan gebeurt? minder contrast? slechtere coronagrafie?]. NCPA originate from misalignments and fabrication errors in the optics downstream of the system’s main wavefront sensor, and therefore are not measured by this sensor. These aberrations evolve on timescales from minutes to hours due to temperature, humidity and gravitational vector changes [dus het draaien van de telescoop?], making them hard to remove with post-processing techniques.
The best place to measure NCPA is the focal plane where the science detector is located, as this completely eliminates the non-common path. Focal-plane wavefront sensing (FPWFS) is concerned with measuring (parts of) the pupil-plane or focal-plane electric field by means of measurements with the science camera.
[kunnen je in deze afbeelding aangeven waar je het over hebt: het stukje helemaal rechts, na de beamsplitter?]
The fundamental challenge in FPWFS is that the currently available detectors measure intensity, instead of electric field. [maar die zijn er wel toch? maar dat gaat waarschijnlijk ten koste van de performance voor de science?] The latter is required for FPWFS, because when you want to reconstruct the pupil-plane wavefront [pupil plane: hoe het licht de telescoop in valt?] from focal-plane measurements, it is necessary to know the full focal-plane electric field. Specifically, this leads to sign ambiguities when trying to measure even phase aberrations. This means that it is impossible to distinguish if an even aberration has a plus or minus sign when observing the PSF. [dit moet je denk ik iets verhelderen, ik dacht meteen ‘wat is een even aberration?’ misschien kun je dat verhelderen met het figuur hieronder?] A focal-plane wavefront sensor is designed to break the sign ambiguity.
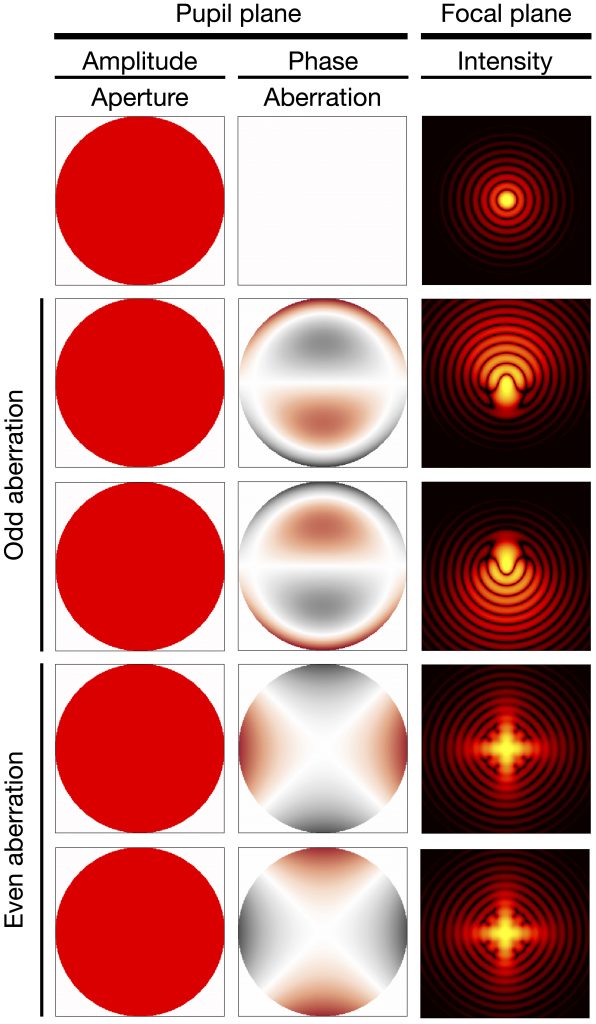
In Leiden we develop novel focal-plane wavefront sensors and test them on-sky [in telescopes?] with state-of-the-art high-contrast imaging instruments. Our goal is to integrate focal-plane wavefront sensing with coronagraphy to increase the efficiency of the system. That means that wavefront sensing measurements can be done simultaneously with science observations and that no telescope time is lost for wavefront sensing.
The Asymmetric Pupil vector-Apodizing Phase Plate coronagraph (APvAPP) combines the vAPP with a pupil-plane amplitude asymmetry [wat betekent dit? zou je kunnen zeggen dat je er een asymmetrisch masker overheen legt?]. The pupil-plane asymmetry breaks the sign ambiguity for even phase aberrations, and allows for simultaneous wavefront measurements and science observations.
[vanaf hier gaat het voornamelijk over algoritmes (behalve de laatste alinea). is het slim dit op een aparte pagina te plaatsen? misschien zelfs combineren met Software? deze pagina vind ik nogal lang zo… je kunt natuurlijk wel makkelijk een verwijzing naar de nieuwe software/algoritme-pagina maken]
Multiple wavefront sensing algorithms can be used to extract the wavefront information from the APvAPP images. We have developed a non-linear wavefront sensing algorithm that fits the aberrations in a physical model of the system to the measured PSF. This algorithm has been successfully tested on-sky using the SCExAO instrument at the Subaru telescope. Multiple instruments at different telescopes host APvAPPs (MagAO-X, SCExAO, ERIS) and can therefore apply this technique.
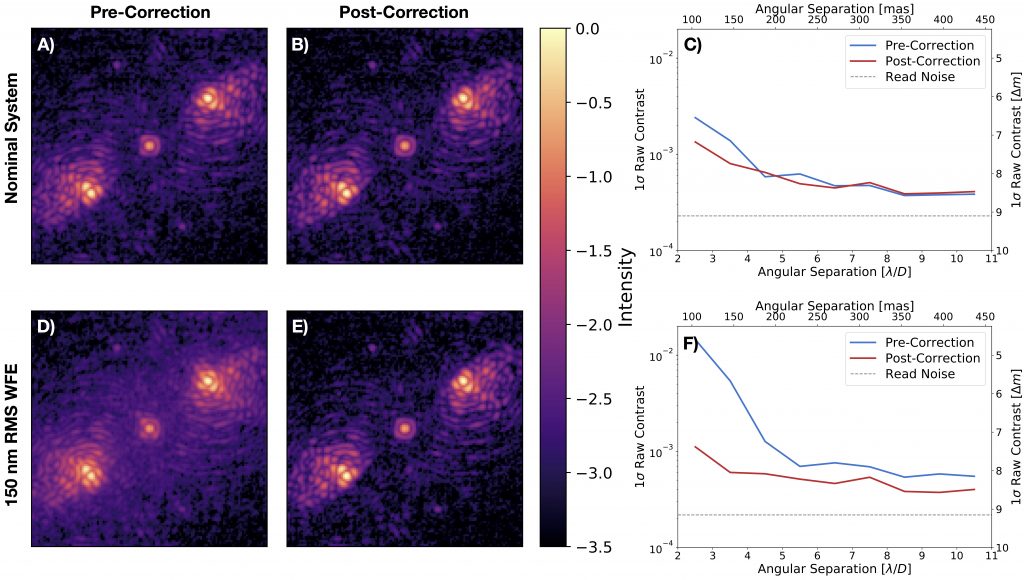
Spatial Linear Dark Field Control (LDFC) is another wavefront sensing algorithm that can be combined with the APvAPP. The goal of this algorithm is to maintain contrast in the dark hole by monitoring the bright field of the vAPP’s coronagraphic PSF. LDFC relies on the linear relationship between wavefront aberrations and focal-plane intensity changes in this bright field. We have successfully implemented LDFC with the APvAPP at SCExAO and tested it on-sky as well.
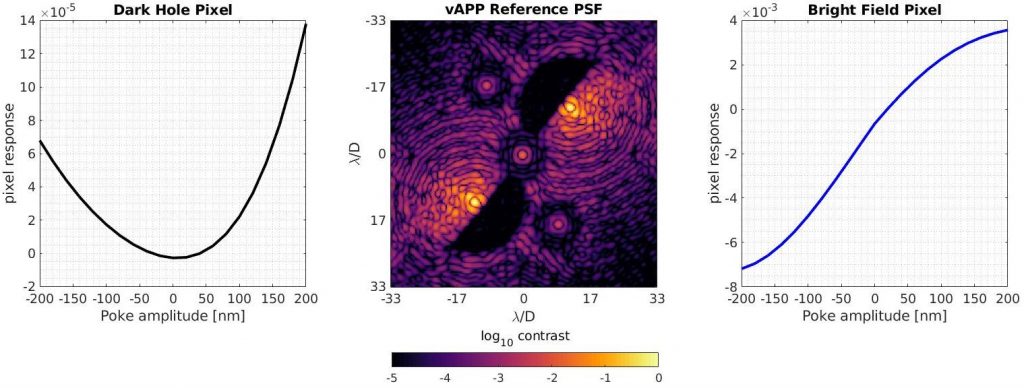
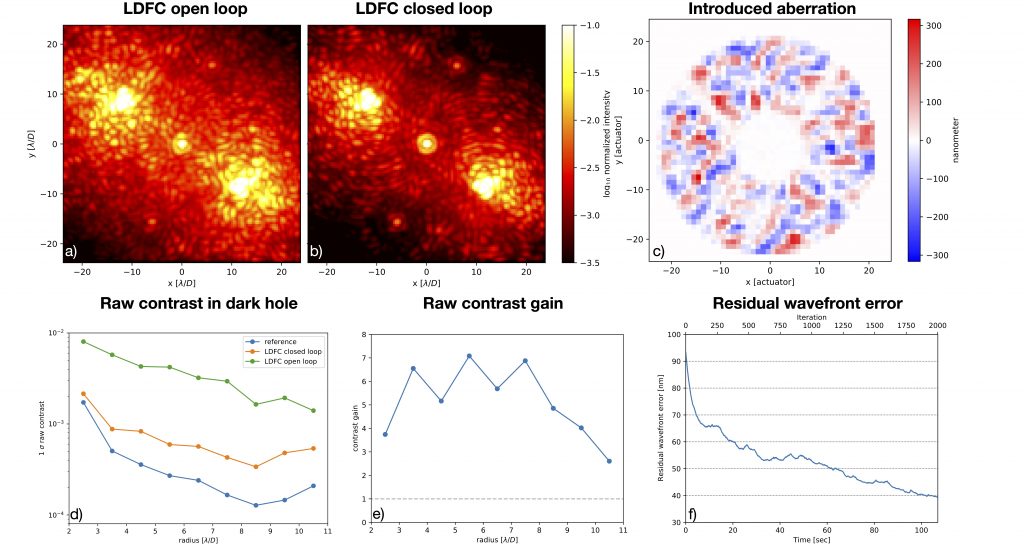
Another FPWFS algorithm is Fast&Furious (F&F). F&F is an iterative wavefront sensing algorithm that that has to run continuously in closed-loop because it uses the previous DM command as diversity to break the sign degeneracy of even phase aberrations. F&F is a robust and numerically simple algorithm that has now been successfully tested on-sky at Subaru/SCExAO and Keck/Nirc2. At Subaru/SCExAO F&F was tested in in context of measuring and correcting the “low-wind effect”.
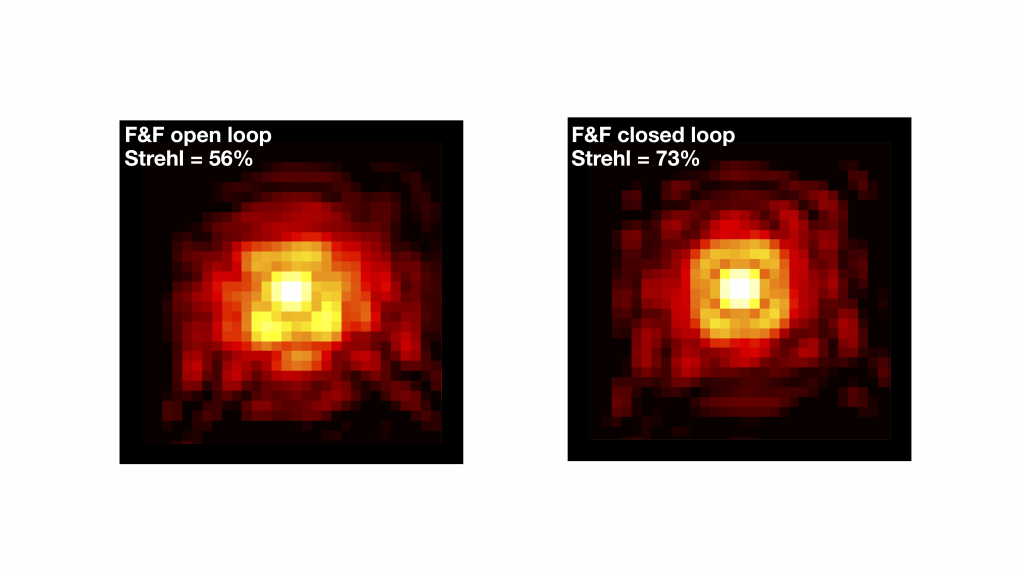
We have also developed another integrated focal-plane wavefront sensor and coronagraph: the polarization-encoded self-coherent camera (PESCC).This is a new and powerful variant of the self-coherent camera (SCC). The PESCC implements a Lyot stop with a pinhole featuring a polarizer, and a downstream polarizing beamsplitter. Due to the implementation with polarization optics, the pinhole can be placed close to the pupil, which relaxes the requirements on the optics size, focal-plane sampling, and spectral resolution compared to the SCC. Furthermore, by placing the pinhole close the the pupil, the PESCC has access to more photons for FPWFS, improving the wavefront sensing performance. The PESCC also enables coherent differential imaging (CDI) as a contrast-enhancing post-processing technique due to its unique design.
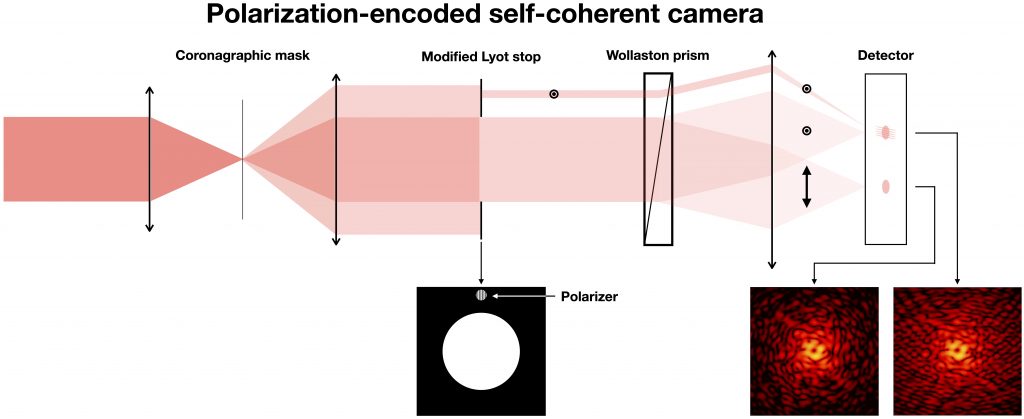
Related Publications
S.P. Bos,A & A 646, A177 (2021) The polarization-encoded self-coherent camera
K. L. Miller, S. P. Bos, J. Lozi, O. Guyon, D. S. Doelman, S. Vievard, A. Sahoo, V. Deo, N. Jovanovic, F. Martinache, F. Snik and T. Currie, A & A 646, A145 (2021) Spatial linear dark field control on Subaru/SCExAO: Maintaining high contrast with a vAPP coronagraph
Vievard, S., Bos, S.P., Cassaing, F., Currie, T., Deo, V., Guyon, O., Jovanovic, N., Keller, C.U., Lamb, M., Lopez, C. and Lozi, J., Adaptive Optics Systems VII. Vol. 11448. International Society for Optics and Photonics, 2020 Focal Plane Wavefront Sensing on SUBARU/SCExAO
Bos, S.P., Doelman, D.S., Miller, K.L. and Snik, F., Adaptive Optics Systems VII. Vol. 11448. International Society for Optics and Photonics, 2020 New concepts in vector-apodizing phase plate coronagraphy
Bos, S.P., Vievard, S., Wilby, M.J., Snik, F., Lozi, J., Guyon, O., Norris, B.R., Jovanovic, N., Martinache, F., Sauvage, J.F. and Keller, C.U., A & A 639, A52 (2020) On-sky verification of Fast and Furious focal-plane wavefront sensing: Moving forward toward controlling the island effect at Subaru/SCExAO
Vievard, S., Bos, S., Cassaing, F., Ceau, A., Guyon, O., Jovanovic, N., Keller, C.U., Lozi, J., Martinache, F., Montmerle-Bonnefois, A. and Mugnier, L., arXiv preprint arXiv:1912.10179 (2019) Overview of focal plane wavefront sensors to correct for the Low Wind Effect on SUBARU/SCExAO
Bos, S.P., Doelman, D.S., Lozi, J., Guyon, O., Keller, C.U., Miller, K.L., Jovanovic, N., Martinache, F. and Snik, F., A & A 632, A48 (2019) Focal-plane wavefront sensing with the vector-Apodizing Phase Plate
Doelman, David S., Fagginger Auer, Fedde, Escuti, Michael J., & Snik, Frans 2018, arXiv e-prints Simultaneous phase and amplitude aberration sensing with a liquid-crystal vector-Zernike phase mask
S. Haffert, 2016, Opt. Express 24 Generalised optical differentiation wavefront sensor: a sensitive high dynamic range wavefront sensor
M. J. Wilby, C. U. Keller, S. Haffert, V. Korkiakoski, F. Snik, A. G. M. Pietrow, 2016, Proc. SPIE 9909, Adaptive Optics Systems V Designing and testing the coronagraphic Modal Wavefront Sensor: a fast non-common path error sensor for high-contrast imaging
van Kooten, Maaike A. M., Doelman, Niek, & Kenworthy, Matthew 2018, Adaptive Optics Systems VI Implications for contrast as a result of the wind vector and non-stationary turbulence